High-throughput experimentation (HTE) has become a core foundation of modern organic chemistry reaction optimisation, with examples now routinely cropping up in the literature.1–4 Whilst the simplest HTE setup requires just a few glass vials and access to Ultra-high Performance Liquid Chromatography-Mass Spectrometry (UPLC-MS), at Domainex, we’ve invested heavily in this approach and provided open access so that all of our chemists/projects can benefit. For example, our glovebox has enabled us to optimise air-sensitive chemistries in plate-based format, a capability that simply wouldn’t have been possible without this acquisition.
Key to our success has been the development of “end-user plates”, where glass vials containing the requisite catalyst are prepared and stored under inert or refrigerated conditions. When a chemist wishes to optimise a project-specific reaction, they remove an end‑user plate from storage (containing these pre-prepared glass vials), add the remaining reagents, and presto! 24 reactions are running with less than one hour of lab work. For our chemistry team, the advantages to end-user plates are three-fold: the individual chemist is saved from tediously weighing out multiple catalysts; the associated templates for experiment write up and reporting make for an expedient, standardised way of working and reporting results; and the small scale (Table 1) of the reactions means that a minimum quantity of precious starting materials are used. As a corollary to these points, there is also a distinct “green” advantage to be gained by conducting reactions on a miniaturised scale, which is substantially smaller than traditional round-bottomed-flask synthesis.
The Domainex end-user HTE platform is based on two commercially available heating blocks, which we colloquially refer to in-house as the “µL” and “mL” size plates. Each come with their pros and cons, and we select the plate size on a case-by-case basis to align with the requirements of the project.
Table 1: Advantages and disadvantages of the µL and mL plate types.
Before using an end-user plate design with project-specific reagents, we validate the design by testing it on a model substrate. We do this to demonstrate that the catalysts are reactive in a plate-based setting and to refine our protocol. For example, we might adjust the recommended order of addition, or the recommended solvents/bases to use. In this blog post, we’ve focussed on our end-user plate for the Suzuki-Miyaura Cross-Coupling (SMCC) reaction to exemplify this technology, the rationale behind the design choices and the validation process conducted in-house.
In building an end-user plate design for the SMCC reaction, we drew inspiration from a number of recently published papers which have carried out plate-based reaction optimisation for the SMCC reaction,4–7 in conjunction with Reaxys’ literature analysis and in-house knowledge. In contrast to the objectives described in each of these sources, we set out with the goal of building a 24-well plate design (rather than a larger 96/384-well design) capable of finding conditions suitable for both traditional singleton synthesis and also library synthesis. We selected a 24-well plate as we believe it offers a balance between diversity of reaction conditions tested and the ease in which a non-HTE specialist could prepare the reactions. By reducing the activation barrier to use, we hope that chemistry project teams are able to run plate-based reaction optimisation as their “first-port-of-call”, with potential long-term benefits in reaction yield and ease-of-purification for the remainder of the project.
During our analysis of the available literature, we observed that the ligands employed were typically either: mono-dentate alkylphosphines (e.g. PCy3), bi-aryl phosphines (e.g. XPhos), or ferrocene derived bis-phosphines (e.g. dppf). Utilising Buchwald Generation 3 pre-catalysts where they were commercially available,8 we selected six pre-catalysts for our plate design. We opted for the Generation 3 pre-catalysts as they are precedented to deliver the required Pd(0) active catalyst on treatment with base, require just a single weighing to obtain both the Pd source and ligand, and are not prohibitively expensive.
Further analysis of the literature found that (corroborating our own experience and in-house ELN dataset), a 4:1 organic solvent/water mixture was often used, where the solvent was typically t‑AmOH, 1,4-dioxane, THF or toluene. The base used was typically an inorganic phosphonate or carbonate. Combining our conclusions, we obtained our 24 well plate design by using 6 palladium pre-catalysts, 2 bases, and 2 solvents (Figure 1). Whilst the identity of palladium pre‑catalysts are fixed, the choice of bases and solvents to use is left up to the chemist who comes to use the end-user plate. We recommend in particular that the choice of solvent prioritises high solubility of the aryl halide and boronic acid in these solvents, as these reactants are added to each vial in the plate by way of stock solutions. Fully dissolved stock solutions are crucial to achieving reliable addition, but the protocol can also tolerate well-mixed, fine suspensions where necessary.
Figure 1: End-user plate design for the Suzuki-Miyaura Cross-Coupling reaction, featuring six different Pd pre-catalysts with space for two solvents and two bases.
Eight end-user plates were prepared in parallel, by making stock solutions of the palladium pre‑catalyst in THF and dosing a sufficient quantity of solution to the appropriate vials. The THF was then allowed to evaporate to give an accurate quantity of pre-catalyst in each vial. Although all the pre-catalysts used are stable to both oxygen and water, the entire process was conducted in a glovebox to provide additional reassurance with regard to batch-to-batch reproducibility of our end-user plates.
To verify that our end-user plate preparation procedure did not interfere with the catalyst performance, we carried out a SMCC reaction using a simple pair of substrates (Scheme 1). To analyse the reactions, we added 2 µmol of N,N-dibenzylaniline as a 4 mM DMSO stock solution to each vial, then diluted the reactions further into a 96-well polypropylene analysis plate. UPLC-MS data was then obtained for each reaction sample, using our optimised 5-95% acetonitrile in aqueous 10 mM ammonium bicarbonate gradient with a two minute run time per sample.
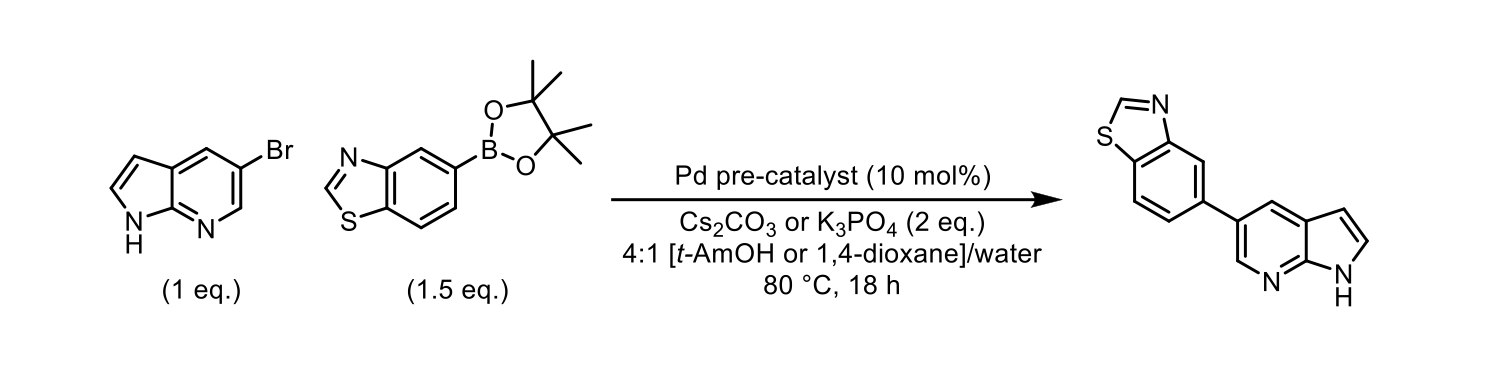
Scheme 1: The model Suzuki-Miyaura cross-coupling reaction used to demonstrate the effectiveness of the end-user plate that was designed.
Analysis of the UPLC-MS data was aided by PyParse, an open-source Python tool designed specifically for the analysis of UPLC-MS from high-throughput experiments.9,10 Among the many advantages that come from “scientist-guided automated data analysis” is the fact that every one of the data points, for every reagent, for every reaction, in every plate, is stored in a standardised format. Long term, our goal is to use this data to build reaction models that can predict the best set of conditions, before even stepping foot in the lab.
The heatmap in Figure 2 is shaded using the “corrP/STD” metric for each reaction: this is the product peak area, divided by the peak area for the N,N-dibenzylaniline, normalised to the maximum observed ratio of product to N,N-dibenzylaniline. Using this metric, “1.0” represents the very best reaction, whereas “0” is where no product formed. By using N,N-dibenzylaniline as an internal standard, we can reliably compare one well with another and mitigate any confounding effects that may arise as a result of using UPLC-MS as an analytical method (e.g. UPLC-silent side reaction products).
Pleasingly, we found that all wells gave conversion to the desired product, with 5/6 pre-catalysts demonstrating extremely high performance. In the case of the less successful pre-catalyst (column 3), we hypothesised that t-BuXPhos Pd Generation 3 was less compatible with this particular substrate. Indeed, we have observed excellent reactivity for this catalyst when using alternative substrates, reaffirming the point that catalyst performance is not easily predicted.
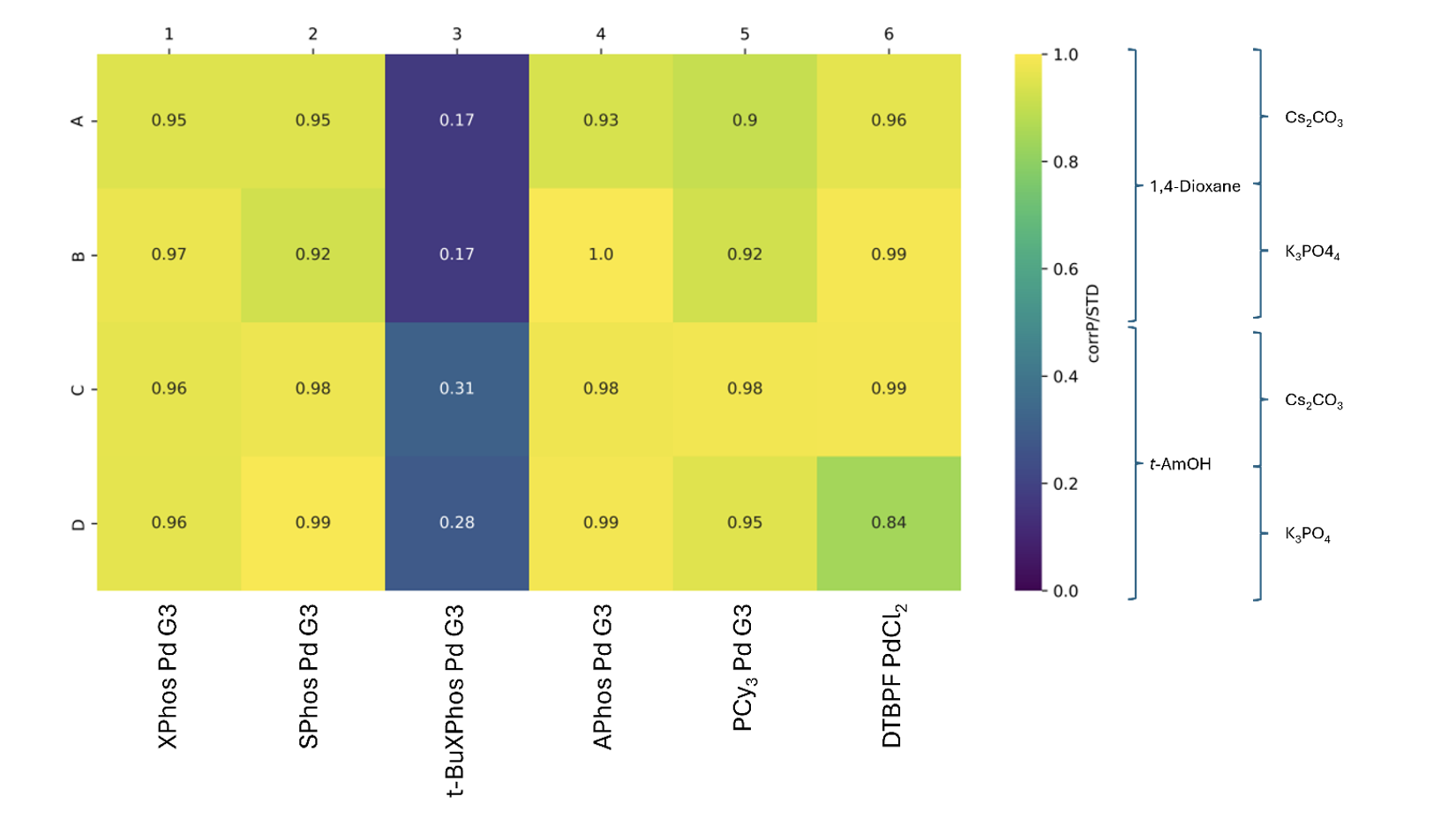
Figure 2: Heatmap for the Suzuki-Miyaura Cross-Coupling reaction performed, as described in Scheme 1.
Scaling up conditions from well D2, ranked amongst the best performing reactions in this plate, an 88% isolated yield was obtained for this bi-aryl product, demonstrating the speed with which a reaction may be optimised using high-throughput experimentation.
Whilst this plate was highly successful, it is, of course, never guaranteed that improved conditions will be found in the end-user plate. However, the 120 minutes spent in reaction design, execution and automated analysis is a small investment, and therefore represents a small risk with a high reward. In cases where no product is generated in any of the 24 wells, we would typically conclude that this disconnection is simply not feasible, or would require such an intensive optimisation campaign as to be prohibitively expensive. Thus, the end-user plates can also allow a project team to “fail fast”, directing them to pursue alternative disconnections before substantial time and money have been invested.
Our SMCC reaction end-user plate design is now available to all chemists, along with a host of other commonly used reaction types such as amide couplings, Buchwald-Hartwig couplings and photoredox transformations. We’re proud of our achievements so far in the HTE space and the impact it has had for our clients, and we continue to “push-the-envelope” in our synthetic capabilities.
- Shevlin, M. Practical High-Throughput Experimentation for Chemists. ACS Med Chem Lett 8, 601–607 (2017).
- Caldentey, X. & Romero, E. High‐Throughput Experimentation as an Accessible Technology for Academic Organic Chemists in Europe and Beyond**. Chemistry–Methods 3, (2023).
- Gesmundo, N. J., Rago, A. J., Young, J. M., Keess, S. & Wang, Y. At the Speed of Light: The Systematic Implementation of Photoredox Cross-Coupling Reactions for Medicinal Chemistry Research. Journal of Organic Chemistry Preprint at https://doi.org/10.1021/acs.joc.3c02351 (2023).
- Townley, C. et al. Enabling synthesis in fragment-based drug discovery (FBDD): microscale high-throughput optimisation of the medicinal chemist’s toolbox reactions. RSC Med Chem 14, 2699–2713 (2023).
- Buitrago Santanilla, A., Christensen, M., Campeau, L. C., Davies, I. W. & Dreher, S. D. P2Et Phosphazene: A Mild, Functional Group Tolerant Base for Soluble, Room Temperature Pd-Catalyzed C-N, C-O, and C-C Cross-Coupling Reactions. Org Lett 17, 3370–3373 (2015).
- Buitrago Santanilla, A. et al. Nanomole-scale high-throughput chemistry for the synthesis of complex molecules. Science (1979) 347, 49–53 (2015).
- Christensen, M. et al. Automation isn’t automatic. Chem Sci 12, 15473–15490 (2021).
- Bruno, N. C., Tudge, M. T. & Buchwald, S. L. Design and preparation of new palladium precatalysts for C–C and C–N cross-coupling reactions. Chem. Sci. 4, 916–920 (2013).
- Mason, J. et al. Automated LC-MS analysis and data extraction for high-throughput chemistry. Digital Discovery 2, 1894–1899 (2023).
- Mason, J., Wilders, H., Fallon, D. & Rianjongdee, F. PyParse. https://github.com/thatchemistryguy/PyParse (2023).